INTRODUCTION
Cadmium is a naturally occurring toxic metal usually found in small quantities in the environment, but human activities tend to significantly increase its levels1. Cadmium is emitted by mining and metallurgical industries, both representing an important source of particles of various sizes, including nanoparticles (NPs), the latter constituting a substantial fraction of aerosol pollution of the workplace2 and ambient air. To human health, Cd and its compounds are carcinogens, mutagens, and teratogens according to the Classification, Labelling and Packaging (CLP) Regulation (EC) No 1272/2008 (Table 1).
Table 1
List of harmonized classification for human health and environmental hazards of Cd compounds according to the CLP Regulation No 1272/2008
Numerous epidemiological studies have demonstrated that high levels of blood cadmium increase the risk of diseases of the cardiovascular system3,4. Even low urinary cadmium concentrations (1 μg/g creatinine) are associated with excess cardiovascular mortality5. However, a Swedish study showed that chronic dietary exposure with the average daily cadmium intake of 17 μg/day was not associated with the risk of cardiovascular diseases (CVD) in women6.
The analysis of published data has shown a positive correlation between the increased blood cadmium and higher blood pressure7 accompanied by the risk of hypertension8. Similar correlations have been demonstrated in in vivo experiments9,10.
Cadmium poisoning increased stroke volume and cardiac output in rats but did not influence the heart rate (HR)11. In a mouse experiment, the end-systolic diameter of the left ventricle (LV) grew excessively while the output fraction decreased, whereas the heart rate, LV wall thickness, and LV end-diastolic diameter remained unchanged12.
In a study conducted on murine cardiomyocytes, the growth of intracellular Ca2+ was suppressed during electrical stimulation. Besides, the maximum shortening and the maximum rate of contraction/relaxation demonstrated a significant decline against the background of stable time parameters of the contraction–relaxation cycle12.
Post-exposure histological studies of the myocardium of mice revealed pronounced Cd-induced interstitial fibrosis manifesting as increased collagen deposition12,13. Structural heart abnormalities were identified in male Wistar rats in a 2-month experiment and included necrotic foci of myocardial fibers, heart tissue hypertrophy, and cardiomyocyte fusion14.
Ultrastructural studies have given evidence of widespread degenerative changes in the cardiomyocytes of rats following chronic cadmium exposure, such as thinning and structure disruption of myofibrils, destruction of mitochondria, enlargement of cisterns and intracellular vacuoles in the sarcoplasmic reticulum11. A study by Chen et al.15 carried out on ventricular cardiomyocytes of Sprague Dawley rats showed that cadmium caused damage to the endoplasmic reticulum and disrupted energy homeostasis.
Incubation of cadmium in human cardiomyocytes obtained from H9 stem cells impaired cell viability, activated apoptosis, increased the number of reactive oxygen species, altered the profile of the action potential, and induced arrhythmia. The MAP kinase signaling pathway was activated as well, whereas p38 MAPK suppression mitigated phenotypic manifestations16. The exposure of HL-1 cells to CdS NPs lowered ATP-dependent luminescence and the frequency of detection of calcium spikes with an increase in NP concentration, while the cardiomyocyte size showed a complex non-monotonic dependence17.
It should be noted that cadmium particles of different sizes pollute the environment by dissolving in environmental media. In our experiment, we studied CdO nanoparticles (CdO NPs), because metal-oxide nanoparticles are the main pollutants in the working area. The composition of the nanoparticles (in terms of the number of atoms) collected from the working area air was found to be about equal to 1:1 of metal:oxygen (on the example of copper)18. So, our objective was to compare the effects of soluble cadmium salts (e.g. CdCl2) and cadmium oxide nanoparticles (CdO NPs) with special attention on the cardiovascular system of rats in a subchronic toxicity study.
METHODS
The experiment was designed and implemented in accordance with the International Guiding Principles for Biomedical Research Involving Animals developed by the Council for International Organizations of Medical Sciences and the International Council for Laboratory Animal Science (2012), and was approved by the Ethics Committee of the Yekaterinburg Medical Research Center for Prophylaxis and Health Protection in Industrial Workers (Minutes Number 2 of 1 February 2018). The experiments were conducted in two series on 2.5-month-old outbred white male rats randomly divided into four groups: CdCl2 exposure and Control group 1, CdO NPs exposure and Control group 2. Each group consisted of 12 animals with an initial body weight of about 220 g. Cadmium compounds were administered intraperitoneally 3 times a week for 6 weeks (up to 18 injections in total).
Experimental doses were chosen by pilot trials to cause adverse shifts in several functional and biochemical indices of the organism’s status but not to induce too heavy an intoxication with a lethal outcome. We maintain that such an animal toxicity model might be regarded as a realistic experimental counterpart of mild-to-moderately expressed occupational intoxications which can be still found in some industries.
For modelled subchronic exposure to cadmium ions, we chose a repeated single dose of the aqueous solution of cadmium chloride 2.5–hydrate estimated on the basis of the median lethal dose: LD50(CdCl2*2.5H2O) = 23.22 mg/kg body weight (b.w.) and equal to 0.38 mg/kg b.w. expressed as cadmium, corresponding to 1/30 LD50. The LD50 value was determined in a previous experiment and was calculated using probit analysis by Shtabsky et al.19 modification. The single exposure dose was limited by the animal’s tolerance to the cadmium chloride solution established in the pilot study.
Such an approach is rarely possible for most metal and metal oxide nanoparticles due to the lack of acute toxicity thresholds. In this regard, the tolerance to cadmium oxide nanoparticles (CdO NPs) was preliminary assessed and a lower single intraperitoneal dose of CdO NPs (0.22 mg/kg b.w., expressed as cadmium) was chosen for the experiment in view of additional limitations related to stability of nanosuspensions.
A stable water suspension of cadmium oxide nanoparticles was obtained by laser ablation of 99.99% pure elemental cadmium target placed under a layer of deionized water. Scanning electron microscopy showed that the nanoparticle shape was spherical with a mean diameter of 57 ± 13 nm.
Control animals received 2 mL intraperitoneal injections of saline (Control group 1) and deionized water (Control group 2) in experiments with CdCl2 and CdO NPs, respectively.
At week 5 of the experiments, electrocardiogram (ECG) and blood pressure parameters were recorded non-invasively in animals without anesthesia using the blood pressure system CODA-HT8 (Kent Scientific, Torrington, USA) and the ecgTUNNEL system (emka TECHNOLOGIES, Paris, France), respectively.
The hemoglobin content was determined using a MYTHIC 18 automated hematology analyzer (C2 Diagnostic, Montpellier, France). Reticulocytes were counted on smears using a light microscope after supravital staining with brilliant cresyl blue.
For blood cadmium level assessment, 1 mL rat blood was diluted three times with concentrated nitric acid. After 2 hours, 2 mL concentrated hydrogen peroxide were added to the sample. After 2 hours, the sample was dried at temperatures up to 400℃. The dry residue was dissolved in 5 mL of distilled water. Blood cadmium levels were measured using the Agilent 7800 inductively coupled plasma mass spectrometer (Agilent, Malaysia).
For genotoxicity assessment, we measured the coefficient of the genomic DNA fragmentation in the nucleated cells of the circulating blood by the random amplified polymorphic DNA (RAPD) analysis as described elsewhere20. Twenty-four blood samples (from 6 rats of each group) were analyzed three times. The degree of DNA migration in the agarose gel at electrophoresis was directly related to the degree of its fragmentation. Non-fragmented DNA (comet head) remained at the same place, while the fragmented DNA (comet tail) migrated. DNA amplification was performed using specific primers and tritiated nucleotides. The applied coefficient of DNA fragmentation was the ratio of radioactivity of the tail to that of the comet’s head.
The biochemical indices determined in the blood serum included albumin content, high density lipoproteins, catalase, ceruloplasmin, natriuretic peptides (NUPs), endothelin-1 (ET-1), and calcium level.
Liver, kidney, and heart tissue sections from four rats of each experimental group were prepared for histologic examination by staining with hematoxylin and eosin. We used the Avtandilov planimetric ocular grid and the Olympus cellSens software (Olympus, Hamburg, Germany) for cellular image analysis.
The ratios of α- and β-myosin heavy chains were determined by gel electrophoresis (SDS–PAGE). The velocity of thin reconstituted filament consisting of actin, troponin, and tropomyosin, sliding over myosin was studied by an in vitro motility assay described previously21,22. Reconstituted thin filament moved on a surface coated with rat myosin in a flow cell in the presence of ATP and calcium ions (pCa=4).
Myocardial contractility was assessed on isolated multicellular myocardium preparations23,24. Thin trabeculae and papillary muscles were dissected from the right ventricle of the same heart. The preparations were fixed to two rods of the length servomotor and force transducer in a thermo-controlled bath (Muscle Research System, Scientific Instruments GmbH, Germany). The experiments were performed in a modified Krebs-Henseleit solution (in mМ: NaCl 118.5; NaHCO3 14.5; KCl 4.2; KH2PO4 1.2; MgSO4 1.2; glucose 11.1, CaCl2 1.9) oxygenated by a mixture of 95% O2 and 5% CO2, pH=7.4 at 35℃. All measurements were taken at the pacing frequency of 2 Hz, temperature of 35℃, and working length of 0.95 LMAX. The recording of isolated muscle contractions was carried out in isometric (Figure 1A) and physiological modes (Figures 1 B and C). The mechanical response under either isometric or physiological mode of contraction was measured using an analogue-to-digital and digital-to-analog converter (PCI–1716S, AdLink Technology Inc., Taiwan) at a frequency of 10 kHz. The force was normalized to the estimated cross-sectional area to obtain mechanical tension values. To estimate force development and relaxation rates, the time course of isometric contraction was normalized to its amplitude.
In the physiological mode, a sequence of loads similar to the physiological sequence in the cardiac cycle was applied to the muscle, which allowed measuring force–velocity relationships (under different afterloads) and force-shortening loops, which resemble the pressure–volume loop typical of the whole heart25,26.
The statistical significance of the intergroup differences was estimated using the Student’s t-test with a correction for multiple testing (ANOVA test) or Mann-Whitney U-test for mechanical characteristics. Differences were considered to be statistically significant at p<0.05.
RESULTS
In both experiments, we modelled moderate subchronic exposure levels and observed statistically significant shifts in a number of rat indices indicating the development of subchronic cadmium poisoning of moderate severity. Toxic effects of cadmium, with a focus on cardiovascular impairments, were described in detail in our previous articles20,22,23,26,27. This study is mainly devoted to a qualitative comparison of the effects of CdCl2 and CdO NPs. We normalized the data in the experimental groups to the same values in the corresponding control groups to compare parameters in different experiments, taking the control values as 1.
Blood cadmium rose following the exposure (Figure 2 A). Cadmium induced a genotoxic effect estimated by the increase in the coefficient of DNA fragmentation in nucleated cells of circulating blood (Figure 2 B).
Hepatotoxic and nephrotoxic effects of cadmium were manifested by the increase in the number of prokaryotic hepatocytes and a decrease in binuclear hepatocytes, as well as by intensified epithelial desquamation and loss of the brush border. These and some other indicators are shown in Figures 2 C–L.
CdCl2 exposure induced a significant heart weight loss (Figure 3 A). The right ventricular cardiomyocyte thickness decreased only after the exposure to CdCl2 (Figure 3 F).
Figure 3
Some cardiovascular indices of rats following subchronic exposure to CdCl2 and CdO NPs (Mean±SD)
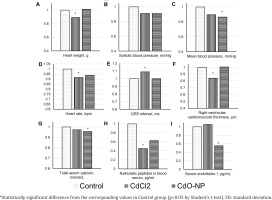
On the electrocardiogram, the QRS complex duration demonstrated an increase with a statistically significant decrease in the heart rate in the CdCl2 group (Figures 3 D and E). Hemodynamic parameters decreased consistently in both experimental groups. The decrease was statistically significant for the mean blood pressure following the exposure to CdO NPs (Figures 3 B and C).
Post-exposure serum calcium decreased, the drop being statistically significant in the CdO NPs group (Figure 3 G); concentrations of vasoactive substances decreased as well [natriuretic peptides in the CdCl2 group and endothelin-1 in the CdO NPs group (Figures 3 H and I)].
The changes in all the above indices following the exposures to CdCl2 or CdO NPs were qualitatively the same, with the exception of ceruloplasmin (Figure 2 H), ET-1 (Figure 3 I), and the right ventricular cardiomyocyte thickness (Figure 3 F).
Following the exposure to CdCl2, we observed a 1.14-fold increase in the percentage of α–myosin heavy chains (MHC) (Figure 4 A) with an appropriate decrease in the proportion of β–MHC. Those changes correlated with a registered 1.38-fold increase in the velocity of thin filament sliding over myosin. At the same time, the exposure to CdO NPs decreased the share of α-MHC by 1.18 times, while increasing that of β-MHC, and the velocity of thin filament sliding over myosin by 1.10 times (Figure 4 A).
Figure 4
Characteristics of myosin (α-myosin heavy chains content and velocity of thin filament sliding over myosin) and contractility of isolated myocardial preparations (Tr, trabeculae; Pm, papillary muscles) in isometric (active tension and time to peak tension) and physiological modes of contraction (maximal isotonic shortening velocity and work produced by isolated muscle preparations).
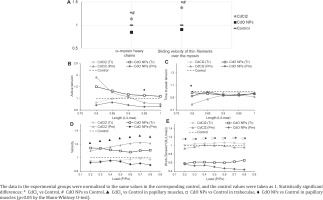
Cadmium reduced the active tension of isolated myocardial muscle isometric contractions (Figure 4 B) except for the curve obtained on trabeculae in the CdCl2 group. The isometric contraction rate curves were slightly above the control one. The curves of time parameters of isometric contraction were slightly below the control one. An example of such reduction in the temporal parameters of isometric contraction is provided in Figure 4 C for the time to peak tension.
The maximal isotonic shortening velocity increased significantly in papillary muscles in the CdCl2 group (Figure 4 D). The same tendency to an increase in the maximal isotonic shortening velocity was observed in the trabeculae of rats following the exposure to CdO NPs. This parameter decreased in the papillary muscles of animals exposed to CdO NPs and in the trabeculae of rats in the CdCl2 exposure group.
Exposure to CdO NPs decreased the amount of work produced by the isolated muscle preparations in both types of muscles (Figure 4 E), while in the CdCl2 exposure group the curves were close to the control one.
DISCUSSION
A 4.2-fold lower blood cadmium level in rats of the CdO NPs exposure group compared to the CdCl2 group might be attributed not only to 1.73-fold smaller single doses of cadmium, but also to the gradual dissolution of nanoparticles. Blood demonstrated compensatory stimulation of erythropoiesis, which was judged by the increase in reticulocytes and the decrease in hemoglobin in both experimental groups (Figures 2 C and D).
The extent of oxidative stress was higher in the CdO NPs exposure group (as shown by low catalase and ceruloplasmin, Figures 2 G and H) and was associated with the ability of NPs to stimulate production of reactive oxygen species (ROS)28.
A typical histomorphometric sign of toxic liver damage was the cadmium-related increase in the number of akaryotic hepatocytes in both experimental groups (Figure 2 I). Judging by the number of binuclear hepatocytes (Figure 2 J), the regenerative capacity of the liver was impaired. This impairment was stronger in the CdCl2 exposure group of animals, possibly attributed to a higher dose of cadmium injected. Toxic liver damage can lead to secondary heart damage due to cross metabolic pathways and circulating inflammation factors29-32.
The brush border loss and epithelial desquamation indicated toxic damage to the renal epithelium (Figures 2 K and L). The gradual dissolution of NPs determined the possibility of their transport as particles in blood flow to organs, particularly to kidneys. NPs accumulated in organs causing excess production of ROS and then dissolved. This might be the reason why we found a more pronounced kidney damage following the exposure to NPs compared to CdCl2. The authors33 came to similar conclusions in an experiment to evaluate the intratracheal effect of Cdcontaining silica NPs. They showed that kidneys proved to be the second target organ after lungs, which was explained by three possible reasons: 1) migration of NPs, 2) circulation of inflammatory factors released from lung, or 3) direct renal action of cadmium ions released from the absorbed Si NPs-Cd. Toxic damage to kidney altered renal hemodynamic function, increased on renin-angiotensinaldosterone system activity, ROS and inflammation. All these factors can cause secondary damage to the heart and blood vessels30,31,34,35.
The decreased heart weight and thickness of right ventricular cardiomyocytes in the CdCl2 exposure group (Figures 3 A and F) may indicate dystrophic changes in the myocardium due to cadmium poisoning. Toxic damage to cardiomyocytes most likely occurred by the mechanisms of apoptosis, since it had been previously shown that Cd2+ can affect apoptotic cell death through a change in the activity of Ca2+/calmodulin-dependent protein kinase II36. There were no such alterations in the CdO NPs exposure group, possibly because of gradual dissolution of NPs and lower blood cadmium in this group.
The revealed electrophysiological disturbances, toxic damage to cardiomyocytes, and a decrease in vasoactive substances (Figures 3 H and I) might weaken the heart pumping function leading to a consistent decrease in blood pressure indices. This finding was more evident in the CdO NPs exposure group and could be linked with a higher extent of oxidative stress and the damaging effect of NPs on both the heart and blood vessels. In the Introduction, we cited many studies in which the increase in the level of cadmium in blood and urine was attributed to the increase in blood pressure. And if we did find a drop in blood pressure, it is important to note that cadmium poisoning does not necessarily increase the blood pressure. For example, arterial hypertension in rats developed only by the 36th week of chronic exposure to cadmium added to drinking water (1 mg%)37. Moreover, hypertension was never registered in rabbits during 9 months of chronic exposure to cadmium in water (20 μg/mL)38. It should be stressed that the meta-analysis by Gallagher and Meliker7, mentioned in the Introduction, led the authors to suppose a negative relationship between urinary cadmium levels and the development of hypertension.
We observed an increasing proportion of α-MHC following the subchronic exposure to CdCl2, and that of β-MHC due to CdO NPs. The latter may be associated with an increase in the number of reactive oxygen species caused by nanoparticles, indirectly (through angiotensin II or ET-1) enhancing the expression of β-MHC39,40.
Cadmium had a negative inotropic effect: it decreased the force of isometric contractions (active tension) in isolated myocardial preparations (Figure 4). It was less expressed following CdCl2 exposure and could be partly explained by a lower extent of oxidative stress.
The maximum rate of force development increased and the contraction-relaxation cycle shortened to compensate for the negative inotropic effect in the hearts of rats in the CdCl2 group (Figures 4 B and C). Temporal parameters of the isometric contraction-relaxation cycle were determined mostly (but not only) by the ratio of myosin isoforms and the rate of processes involved in calcium regulation of contractions41,42. In both experimental groups, we observed a decrease in temporal parameters of isometric contraction accompanied by a slight increase in the maximal rate, while at the molecular level we noted a rise in the proportion of β-MHC following CdO NPs exposure compared to the controls in contrast to the increased share of α-MHC due to CdCl2. It appears that the ratio of α- and β-MHC depends on the type of Cd preparation (CdCl2 solution or CdO NPs suspension) while the force, rate, and time changes in isometric contraction in multicellular myocardial preparations follow the same patterns. This means also that cadmium has a strong influence on the rate of processes involved in calcium regulation of contractions, which may be explained by the notorious cadmium/calcium antagonism43, as well as the cadmium ability to mimic calcium36.
Alterations in the maximum isotonic shortening velocity in the papillary muscles (Figure 4 D) correlated with the changes in the ratio of α- to β-MHC (Figure 4 A). The velocity increased with CdCl2 exposure and rising share of α-MHC, and decreased with CdO NPs exposure and increasing proportion of β-MHC, while trabeculae demonstrated the opposite trend. Such a difference between trabeculae and papillary muscle data might be related to different functions and locations of trabeculae and papillary muscles in the whole heart. The former is located on the ventricular wall and are involved in its expansion and contraction. The latter are located in the cavity and hold the tricuspid valve, thus experiencing high loads during the work of the heart.
The toxic effect of nanoparticles reduced the efficiency of the myocardial contractile function, which was assessed by the work produced by isolated muscles (Figure 4 E). It seems to be associated with the ability of NPs to initiate the formation of a large number of ROS and a gradual (longterm) dissolution of NPs in the body. In contrast, the work did not change in the CdCl2 group, possibly owing to a lower oxidative stress mentioned above.
Summarizing the above, Cd as a soluble salt (CdCl2) or oxide nanoparticles (CdO NPs) had a general toxic, hepatotoxic and nephrotoxic effects. These effect directions were the same with the exception of ceruloplasmin and endothelin-1. At the same time, heart force and velocity characteristics of isometric contraction changed similarly due to cadmium toxicity in both experimental groups. This means that cadmium reduces the isometric contraction force, increases velocity parameters of isometric contraction and decreases the temporal ones. Qualitative differences were found in the α- and β-MHC content, the velocity of thin filament sliding over myosin, the maximum isotonic shortening velocity of isolated multicellular preparations, and the work performed by the muscles. The observed differences may be related to increased oxidative stress, a large number of ROS and a large level of inflammation caused by NPs.
Limitations
We acknowledge methodological limitations in our study. For a qualitative comparison, we used data of two successive experiments on outbred rats from different litters. Single doses of CdCl2 and CdO NPs expressed as Cd differed by a factor of 1.73. Mechanical and electrophysiological processes in the work of the heart differ in small rodents and large mammals; thus, caution should be taken when extrapolating our findings from animals to humans.
CONCLUSIONS
Our study showed moderate cadmium toxicity in rats following subchronic exposure to the CdCl2 solution and CdO NPs suspension, as established by hematological changes, shifts in blood serum biochemical indices, and histomorphometric alterations in liver and kidney tissues. Oxidative stress was stronger in the animals exposed to CdO NPs than in the CdCl2 exposure group.
In both experimental groups, cadmium poisoning induced a decrease in hemodynamic parameters. We observed a negative inotropic effect on isolated muscle myocardium preparations. We established qualitative differences between the effects of CdO NPs and CdCl2 at the molecular level in α- or β-myosin heavy chains and in the velocity of thin filament sliding over myosin. We believe that the molecular changes were the reason for the revealed qualitative differences in toxic effects of cadmium between CdO NPs and CdCl2 exposure groups at the multicellular myocardial level. It is important to note that the exposure to CdO NPs impeded the work produced by the muscles, thus indicating the impaired efficiency and compensatory capabilities of the myocardium induced by nanoparticles.